How Does The Cellular Response To Egf Result In Tissue Repair
Abstract
To combat the various DNA lesions and their harmful effects, cells accept evolved different strategies, collectively referred as DNA damage response (DDR). The DDR largely relies on intranuclear protein networks, which sense DNA lesions, recruit DNA repair enzymes, and coordinates several aspects of the cellular response, including a temporary cell wheel arrest. In addition, external cues mediated past the surface EGF receptor (EGFR) through downstream signaling pathways contribute to the cellular DNA repair capacity. Withal, jail cell cycle progression driven past EGFR activation should be reconciled with jail cell cycle arrest necessary for effective DNA repair. Here, we show that in damaged cells, the expression of Mig-6 (mitogen-inducible gene 6), a known regulator of EGFR signaling, is reduced resulting in heightened EGFR phosphorylation and downstream signaling. These changes in Mig-six expression and EGFR signaling practise not occur in cells deficient of Mre-11, a component of the MRN complex, playing a central function in double-strand break (DSB) repair or when cells are treated with the MRN inhibitor, mirin. RNAseq and functional analysis reveal that Deoxyribonucleic acid harm induces a shift in prison cell response to EGFR triggering that potentiates DDR-induced p53 pathway and cell bicycle arrest. These data demonstrate that the cellular response to EGFR triggering is skewed by components of the DDR, thus providing a plausible explanation for the paradox of the known role played by a growth cistron such as EGFR in the DNA damage repair.
Introduction
Cells are continually exposed to DNA damaging agents of endogenous (metabolic) and environmental origin. Double-strand breaks (DSBs) threaten chromosomal integrity and must be repaired efficiently to maintain cell viability and to prevent malignant transformation. Diverse and complex molecular mechanisms, collectively termed the DNA damage response (DDR), have evolved to alleviate the consequences of genotoxic damage and to maintain tissue homeostasis. The DDR largely relies on intranuclear protein networks, which sense Deoxyribonucleic acid lesions and recruit DNA repair enzymes.
The Mre-11–Rad50–Nbs1 (MRN) and the Ku heterodimer (Ku70-Ku80) complexes are amidst the 'first responders' that bind to DSBs independently of other factors. These complexes play important roles in sensing and signaling as well as promoting the repair of Dna breaks via both homologous recombination (Hour) and not-homologous end-joining (NHEJ)1,2,3,4,v. MRN and Ku70/fourscore complexes play fundamental roles in recruiting and activating the kinases ataxia-telangiectasia mutated (ATM) and Dna-dependent protein kinase catalytic subunit (Dna-PKcs), respectively6,seven,viii,nine. In turn, these phosphoinositide-3-kinase (PI3K) family unit members phosphorylate other proteins including the histone H2AX (referred to as γH2AX) over a large region surrounding the DSB, leading to readily visualized foci, and creating binding sites for other DDR proteins5,10.
While DDR is essentially a cell-autonomous process that occurs in the nucleus, the cell surface epidermal growth factor receptor (EGFR) has been shown to accelerate DSB rejoining. The EGFR itself (upon nuclear translocation) or by signaling through ERK and AKT, regulates the phosphorylation, distribution, and action of key proteins in the DDR pathway (reviewed in11). We recently demonstrated that macrophages, via the release of heparin-bounden EGF-like growth factor (HB-EGF), facilitate DSBs repair in EGFR expressing neighboring cells12, consistent with the notion that Dna harm is controlled, in part, by extracellular cues.
The EGFR belongs to the ErbB family of receptor tyrosine kinases (RTKs), exerts critical functions in cell physiology, and is frequently mutated and/or overexpressed in man cancerthirteen,14,15.
Deregulated EGFR signaling in cancer has been linked to resistance to chemo- and/or radiotherapy every bit well every bit increased Dna harm repair chapters, whereas inhibition of the EGFR attenuates Deoxyribonucleic acid damage repairsixteen,17,xviii,nineteen. Of course, beyond effects related to Dna repair, ErbB family unit members including the EGFR itself are master regulators of jail cell cycle progression and survival in multiple prison cell types including fibroblasts and epithelial cells14,xv,20.
While numerous reports have demonstrated that EGFR signaling is playing a role in Deoxyribonucleic acid harm response, piddling is known how the diverse effects of EGFR activation are coordinated in cells challenged past DNA damaging agents. Specifically, it is reasonable to assume that cell-cycle progression driven by EGFR activation may annul the cell-cycle arrest necessary for effective DNA repair to occur. We hypothesize that Dna damage modifies indicate transduction triggered past EGFR activation. We farther advise that these 'inside-out' signaling events serve an essential role in synchronizing cell-cycle progression and cell survival mechanisms with DDR to optimize atmospheric condition for cell recovery and Dna damage repair.
Results
DNA harm enhances ligand-dependent EGFR-induced signaling
To determine whether Dna impairment regulates EGFR signaling, we monitored HB-EGF-induced EGFR-signaling events in cells after bleomycin treatment (Fig. S1C and21,22) and compared them to HB-EGF-treated controls. Normal human dermal fibroblasts (HF) pretreated with bleomycin and subsequently exposed to HB-EGF exhibited higher levels of pEGFR (Y1173; on average threefold higher, n = ix), pAKT and pERK signals when compared to cells treated with HB-EGF alone (Fig. 1A). Similar results were obtained when DNA impairment was induced by peroxide (Fig. S1A,B). Moreover, HB-EGF handling of mock-treated fibroblasts induced short-term EGFR phosphorylation, which returned to baseline levels within ten min, whereas fibroblasts pretreated with bleomycin and afterwards exposed to HB-EGF exhibited higher levels of pEGFR for an extended fourth dimension of upward to thirty min (Fig. 1B).
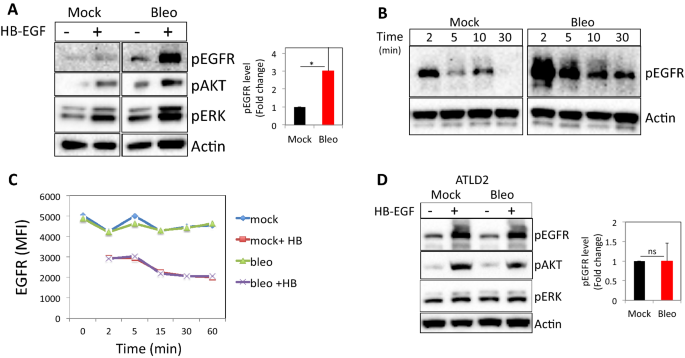
DNA damage enhances ligand-induced EGFR phosphorylation and signaling. Normal human dermal fibroblasts (HF) were either left untreated or treated with bleomycin for xxx min, followed by all-encompassing washing and subsequent exposure to HB-EGF (10 ng/ml). Afterwards 5 min (A) or at indicated time points (B), cells were lysed. Western blot analyses were performed on prison cell extracts using antibodies to phosphorylated EGFR (Y1173), phosphorylated AKT, and ERK. Anti-β-actin immunoblotting revealed relative amounts of protein in each lane. (C) HF were treated as above and at the indicated fourth dimension points cells were collected and immune-stained for jail cell surface expression of EGFR. MFI: Median fluorescent intensity. (D) Fibroblasts from an ATLD patient (ATLD2) were treated and analyzed as in (A). pEGFR signal intensities were quantified and normalized to actin signal (loading command). The level of EGFR phosphorylation in response to HB-EGF treatment in bleomycin treated cells as compared to that of mock-treated cells (set equally 1) are shown (A: n = 9; D: north = four). Representative results of at least three independent experiments are shown. *p < 0.05; ns: non-significant.
Using an EGFR phosphorylation antibody assortment, we simultaneously assessed the relative levels of phosphorylation of 17 dissimilar sites for the human EGFR family23. The preferential phosphorylated site upon HB-EGF stimulation was Y845. Moreover, ErbB2Y1112 and ErbB2Ser113 were also phosphorylated upon HB-EGF treatment, just to a lesser extent in bleomycin-treated cells. On the other paw, HB-EGF-induced phosphorylation of site Y1173 was ~ threefold higher in bleomycin-treated cells every bit compared to control cells, verifying the above findings (Fig. S1C).
Activation of cell surface receptors triggers regulatory processes that restrict the elapsing of signaling via ligand-induced internalization. 1 possible caption for EGFR enhanced and prolonged stimulation in DNA damaged cells might be a higher number of surface receptors, partially due to incomplete ligand-induced internalization. To test this possibility, we monitored EGFR surface expression in fibroblasts that were either left as control or treated with bleomycin at various fourth dimension points after HB-EGF incubation. Of note, as shown in Fig. 1C, there was no significant divergence in the level of cell surface EGFR in control vs bleomycin-treated cells with a similar extent of HB-EGF-induced receptor downregulation (Fig. 1C).
Next, we used fibroblasts from a patient with clutter telangiectasia-like disorder (ATLD), a human syndrome with hypomorphic mutations in Mre-eleven24. This cell line (kindly provided by Y. Shiloh from Tel Aviv University) was used to decide whether the DNA damage-associated increase in EGFR activation is dependent on sensing Deoxyribonucleic acid harm by the MRN pathway. ATLD2 cells responded ordinarily to HB-EGF with increased pEGFR, pAKT, and pERK. However, in dissimilarity to wild-type HF (Fig. 1A), in ATLD2 cells treated with bleomycin, the level of HB-EGF induced EGFR, AKT, and ERK phosphorylations were not elevated (Fig. 1D). These results suggest that Mre-11 is required for increased EGFR activation by exogenous ligands in the context of Deoxyribonucleic acid damage.
Pharmacological inhibition of Mre-11 inhibits EGFR activation but in cells suffering from Deoxyribonucleic acid damage
To confirm the role of the Mre-11 and MRN complex in DNA damage-controlled EGFR activation, we assessed the consequence of mirin25 on ligand-induced EGFR activation in normal fibroblasts. Mirin is an inhibitor of Mre-11 exonuclease activity that prevents MRN-dependent activation of ATM25,26. Notably, mirin prevented HB-EGF-induced phosphorylation of the EGFR and ERK in bleomycin-treated (Fig. 2A). In dissimilarity, treatment with the inhibitors blocking either ATM (Ku55933) or Deoxyribonucleic acid-PK (Nu7026) had simply pocket-sized furnishings on the specific activation induced by HB-EGF (Fig. S2A). Similar results were obtained in peroxide-treated cells (Fig. S2B,C). Moreover, immunofluorescence shows translocation of phosphorylated ERK to the nucleus upon HB-EGF treatment, which is significantly reduced when cells are treated with mirin (Fig. S2D).
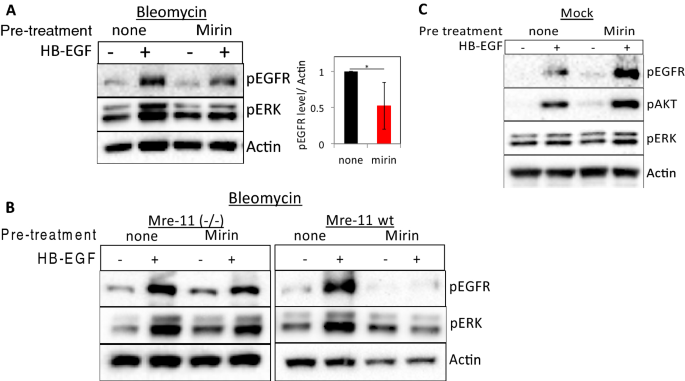
Pharmacological inhibition of Mre-11 interferes with ligand-dependent EGFR signaling in cells suffering from Deoxyribonucleic acid damage. (A) HF were either left untreated or treated with mirin (100 µM) for one hr and then were treated with bleomycin for an additional 30 min followed by HB-EGF (x ng/ml) handling for 10 min. (B) ATLD2 cells (Mre-11−/−) or ATLD2 cells reconstituted with wild type Mre-xi (Mre-11 wt) were treated as in (A). (C) HF were either left untreated or treated with mirin for ane hour and then left untreated (mock) and HB-EGF was added for 5 min. Cells were lysed and subjected to Western absorb analyses using the indicated antibodies equally above. pEGFR signal intensities were quantified and normalized as in Fig. ane (due north = 5). Representative results of at to the lowest degree iii independent experiments are shown.
To dominion out off-target effects of mirin, we additionally used ATLD2 cells. As expected, in Mre-11−/− ATLD2 cells mirin (that human action by binding to the agile site of the Mre-11) had no consequence on HB-EGF-induced EGFR signaling (Fig. 2B, left panel). All the same, when these cells were reconstituted with wild-type Mre-eleven27, the effect of mirin on ligand-dependent EGFR signaling was recovered (Fig. 2B, correct panel), suggesting that the inhibition is specific for Mre-11 and not merely inhibiting EGFR activation. Moreover, the effect of mirin on EGFR, AKT, and ERK phosphorylation was specific for Dna damaged cells as no inhibition of HB-EGF-induced EGFR-signaling was observed in mock-treated controls (Fig. 2C).
Taken together these data revealed a link betwixt nuclear Mre-eleven in cells suffering from Deoxyribonucleic acid damage and ligand-induced activity of surface EGFR.
Regulation of Mig-6 expression past Deoxyribonucleic acid damage
Mig-six is a cytoplasmic poly peptide that functions as a feedback negative regulator of the EGF receptor-family28. Chiefly, Mig-6 knockdown led to a pregnant increase in pEGFR (Y1173) as well as in pAKT and pERK in response to EGF treatment, whereas reconstitution of Mig-half dozen expression attenuated the activation of EGFR and the downstream signaling pathway29,30. Hence, we reasoned that Mig-half-dozen might mediate the regulation of EGFR signaling by DNA damage. Consequent with this assumption, Mig-6 RNA expression was markedly reduced in fibroblasts following bleomycin treatment (Fig. 3A) but not in ATLD2 cells (Fig. 3B), which corresponded with the level of EGFR phosphorylation in these cells (Fig. 1). Moreover, Mig-6 downregulation was abrogated in mirin-treated cells. Although non statistically significant, Mig-6 was even elevated following bleomycin treatment (Fig. 3C) suggesting that upon Deoxyribonucleic acid damage, Mre-11 may be responsible for the decrease in Mig-half-dozen expression and consequently enhanced EGFR activation.
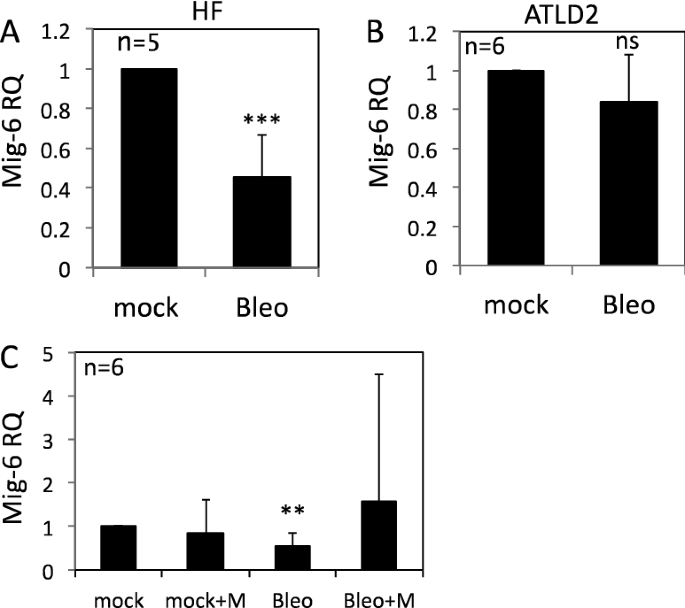
Regulation of Mig-6 expression by DNA damage. HF cells (A), ATLD2 cells (B), or HF cells that were pre-treated with mirin (100 µM) for an hr (C), were either left untreated or treated with bleomycin for 30 min and then RNA was extracted. Mig-6 mRNA expression level was determined by qRT-PCR and normalized to GAPDH. Graphs testify an average (± STD) of the indicated number of contained experiments in each group. The expression of Mig-6 in control cells was set every bit one. **p < 0.005; ***p < 0.0001; ns: non-significant.
DNA damage alters HB-EGF-induced gene expression profile
To test how DDR skews cellular response to EGFR activation, we assessed gene expression later treatment with HB-EGF following bleomycin mediated Dna damage in comparison to controls treated with HB-EGF solitary. RNA extracted from cells treated with HB-EGF for xxx and 90 min were subjected to RNA sequencing (RNA-seq; Fig. S3A).
Overall, RNA-Seq assay identified more than differentially expressed genes (DEG) following bleomycin treatment. A total of 7 and 45 DEG were identified between 30 and xc min in mock and bleomycin treated cells, and 48 and 146 DEG were identified in response to HB-EGF treatment in the two groups, respectively (Fig. S3B). Significantly, only a pocket-size overlap between genes differentially expressed in response to HB-EGF could be observed (Fig. S3C), suggesting that EGFR triggering induces expression of different factor sets in damaged cells every bit compared to mock treated controls. Indeed, Canonical Ingenuity Pathway Analysis (IPA) of DEG at 90 min and Gene set enrichment analysis (GSEA) revealed a articulate divergence in signaling pathways activated by HB-EGF in the context of DNA harm as compared to control cells (Figs. 4A and S3D). These analyses revealed that HB-EGF treatment in cells suffering from Deoxyribonucleic acid damage reinforced the activation of Deoxyribonucleic acid harm repair, UV response, G2/Grand Deoxyribonucleic acid damage checkpoint, PI3K/AKT signaling and p53 pathways. On the other hand, cell cycle related pathways (such as mitotic spindle and G2 checkpoint) were reduced (Figs. 4A and S3D–F). Of note, the interferon-blastoff response pathway induced by HB-EGF in bleomycin-treated cells was recently associated with p53 activation31.
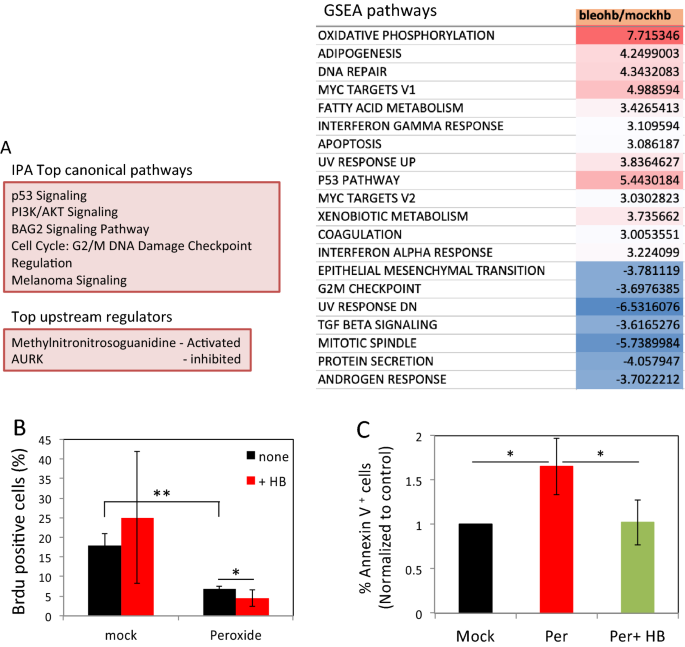
Deoxyribonucleic acid damage alters gene expression profile and cellular response to HB-EGF triggering. (A) HF were treated as in Fig. 1A. Subsequently xxx and ninety min RNA was extracted and was subjected to RNAseq analysis. Differentially expressed genes contradistinct with college than twofold changes were selected. A list of IPA canonical pathways that are enriched in bleomycin treated cells stimulated with HB-EGF compared with those from HB-EGF stimulated mock cells (upper left panel). IPA upstream functional analysis was used to predict the superlative upstream regulators from differentially expressed genes (lower left console). Gene fix enrichment analysis (GSEA) pathways that are up-regulated (red) or downwardly-regulated (blueish) in response to HB-EGF treatment in bleomycin as compared with mock-treated cells (right panel). (B) Mock and peroxide (500 µM) treated cells were pulsed with BrdU for two hours immediately afterwards treatment. After 24 h the cells were fixed and analyzed by flow cytometry. The per centum of BrdU positive cells is shown. Representative results of iii contained experiments are shown. (C) Cells were treated as to a higher place and after 12 h were collected and stained with Annexin-Five. The percentage of Annexin-V positive cells of three independent experiments was normalized to mock (fix equally 1). *p < 0.05; **p < 0.005; ns: non-significant.
To validate these RNAseq findings relating to prison cell cycle abort, nosotros analyzed 5-Bromo-2′-deoxyuridine (BrdU) incorporation into replicating Dna in cells suffering from DNA damage to examine the effect of HB-EGF on cell-cycle arrest. We specifically used peroxide as this treatment induces a high number of DNA breaks and causes complete block in prison cell-cycle12. In the kickoff two hours, a turn down in BrdU incorporation is observed in peroxide treated cells as compared to command cells. Consistent with the RNAseq analysis, this decline in BrdU incorporation is further intensified in the presence of HB-EGF (Fig. 4B).
Later Dna damage the cell wheel is blocked to allow time for repair. Nonetheless, depending on the extent of Dna damage, apoptosis can exist induced (reviewed in32). Therefore, we tested the effect of EGFR triggering on Dna damage-induced prison cell decease using Annexin-V staining. The percentage of Annexin-V positive cells increased significantly following peroxide treatment as compared to mock-treated cells, peaking at 12 h later treatment (Fig. S3G). Interestingly, HB-EGF rescued cells from peroxide-induced cell death (Figs. S3G and 4C).
Overall these results demonstrate that the cellular response to EGFR triggering is skewed in cells suffering from DNA harm and is characterized with augmented DNA harm response, p53 pathway, and a transient cell-cycle arrest. These changes are necessary for effective Dna repair and are consistent with improved DSB resolution in HB-EGF treated cells12.
Discussion
Post-obit Dna damage, cells activate the DDR pour that largely relies on intra-nuclear protein networks, sensing DNA lesions and analogous Deoxyribonucleic acid repair. The Mre-11-RAD50-NBS1 (MRN) complex is one of the kickoff sensors of DNA harm that plays a crucial role in orchestrating DNA harm response8. The DDR also communicates with the cell-cycle mechanism and induces a transient p53-mediated cell-cycle abort, which provides time for DNA repair33.
In addition to its pro-proliferative activity, EGFR has been shown to enhance DDR signaling and to facilitate DNA damage repaireleven,12. However, the question arises as to how the ii conflicting activities of cell-cycle progression driven past EGFR and the growth arrest necessary for effective Deoxyribonucleic acid repair tin can be reconciled. It is reasonable to assume that stress conditions, such as DNA damage, within a cell may crave a rapid and flexible strategy of diversifying the response to external stimuli. Our analysis demonstrates how such scenario tin can be generated, namely DDR modifies ligand-induced EGFR-ERK-AKT signaling pathway (Fig. 5).
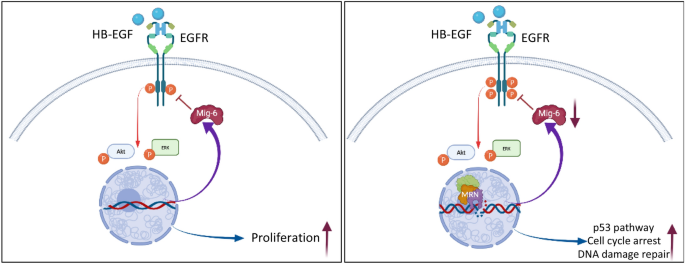
Model furnishings of DNA harm and repair on EGFR signaling and cellular response. (A) Under homeostatic conditions binding of ligand to EGFR induces canonical signals supporting cell proliferation and survival. (B) Upon induction of DNA harm, the MRN complex is assembled around sites of DSBs leading to a decrease in Mig-half dozen expression and amplifies ligand-induced EGFR signaling. In turn, amplified EGFR signaling enhances DDR-induced p53 pathway and cell cycle arrest and facilitates DSBs rejoining. Images created using BioRender.
Our study reveals that the DDR pathway biased ligand-induced EGFR-signaling network and cistron expression profiles, which may provide an adaptive reward for DNA-damaged cells. This novel pathway depends on intact MRN complexes affecting the expression of Mig-six to coordinate Deoxyribonucleic acid damage repair with response to extracellular signals. We advise that this bidirectional crosstalk of nuclear (DDR) and extracellular signals (EGFR) is required to synchronize and coordinate cell-cycle arrest and Deoxyribonucleic acid repair.
Specifically, our data demonstrate that ligand-induced EGFR phosphorylation and two well-characterized downstream signaling pathways, ERK and AKT, are enhanced in cells suffering from DNA damage. Importantly, Dna impairment modulates the biological responses to ligand-induced EGFR signaling. In contrast to the pro-proliferative activity of EGFR signaling, triggering this pathway in cells suffering from Deoxyribonucleic acid impairment augments the canonical Dna harm-induced p53 pathway and reinforces transient cell growth arrest and efficient Dna damage repair. Notably, a recent report has demonstrated that upon DNA damage ATM stabilizes the transforming growth gene β (TGFβ) receptor, which in plough execute its anti-proliferative activity and thus contribute to cell wheel abort34. However, our study presents an even more than complex scenario, whereby DNA damage modifies EGFR signaling and changes the cellular response to EGFR triggering, strengthen p53 and transient jail cell cycle arrest. Interestingly, several studies have demonstrated that in subsets of cancer cells, and specifically breast cancer cells, EGFR function changes from proliferative in master tumors to growth-inhibitory and apoptotic in metastatic tumors35,36,37,38,39. Moreover, a biased downstream ligand-induced EGFR signaling and the activation of STAT1 were shown to be responsible for enhanced apoptosis and growth inhibition in the metastatic cellsforty.
The variety of signaling networks activated by ligand-dependent activation of EGFR prevents the logical elucidation of how the biochemical signal links to the appropriate biological responses. All the same, it would be reasonable to argue that the duration and strength of signals that are tightly regulated by the action of numerous negative regulatory mechanisms, such as Mig-6, is translated into different gene expression profiles that could convert cellular function. A previous report suggested that unbalanced expression levels of Mig-six with relation to EGFR lead to persistent activation of EGFR that initiates a pro-apoptotic cascade39.
Upon ligand binding, Mig-6 associates with the intracellular domain of EGFR and inhibits EGFR signaling via two mechanisms that involve receptor deposition and kinase suppression28,41,42,43. Thus, reduced Mig-6 is expected to confer higher sensitivity to EGFR triggering. Our data demonstrate that DNA damage reduces Mig-6 expression and heightens EGFR phosphorylation and activation. Chiefly, these DNA damage-dependent modifications in ligand-induced EGFR activation did non involve whatever changes in EGFR surface expression, equally would be expected from a mechanism involving receptor degradation.
The regulation of EGFR activation by DNA damage was dependent on the expression of Mre-11 and presumably on the assembly of an active MRN complex upon DNA damage. Mre-11 is an of import component of the Mre-11-RAD50-NBS1 (MRN) complex that plays a crucial role in DDR, equally it is i of the first sensors and responders to DNA damage. Through interacting with other players at the double-strand intermission, the MRN complex initiates and amplifies DDR signaling and orchestrates Deoxyribonucleic acid damage repair pathways44,45. In add-on, to produce the advisable biological responses Dna damage tin positively regulate transcription initiation of specific transcriptional programs46,47,48. Interestingly, we observed a change in Mig-vi mRNA levels shortly later on the consecration of DNA damage, thus coupling DDR role to signaling through cell surface receptors. A previous study demonstrated that ligand-induced activation of the EGFR is lacking in ataxia-telangiectasia (A-T) cells49, suggesting a role for ATM in EGFR activation. Similarly, ATM has been shown to play a function in the insulin receptor-signaling pathway that controls translation initiationl. However, in contrast to our findings, these cytoplasmic functions of ATM in regulating surface receptor signaling are taking place in control cells and are independent of its function in DNA damage.
In summary, we described an 'within-out' signaling, originating from the Deoxyribonucleic acid lesion to modify signal transduction triggered past EGFR activation. This allows the cells to survive and to perform efficient DNA repair. Thus, this study provides a general framework in which cells re-wire cellular networks in response to external cues mediated by surface receptors co-ordinate to their cellular condition.
Materials and methods
Cells
Main normal man dermal fibroblasts (HF) were provided by the Department of Surgery, Hadassah Hebrew-University Medical Center, Jerusalem, Israel. ATLD2 fibroblasts and ATLD cells complemented with wild-blazon Mre-eleven (a generous gift from Dr. Yosei Shiloh, Tel Aviv Academy, Israel). Cells were maintained (not more than than 10 passages) in a loftier-glucose DMEM medium (Life Technologies, Grand Island, NY). All media were supplemented with 10% rut-inactivated fetal dogie serum, 1% sodium pyruvate, and 1% penicillin/streptomycin (Biological Industries, Beit-Haemek, Israel) at 37 °C and 5% COii.
Experimental protocol
The in-vitro experimental protocol is adapted from our previous work12. For Dna damage consecration cells were treated with either bleomycin (xv µg/ml) or hydrogen peroxide (EMD Millipore Corp. Billerica, MA; 500 µM H2O2 in PBS) or were mock-treated for 30 min, and were so done with fresh media. Only at that stage, HB-EGF (PeproTech, Rocky Hill, NJ; 10 ng/ml) was added, and cells were incubated for the indicated time points.
Cell lysis and immunoblotting
Cells were lysed with X3 LDS-Page sample buffer (GeneScript, Piscataway, NJ). The lysates were boiled for 30 min so H2O (one:3 five/v) was added and samples were boiled for an additional 5 min. Samples were kept at − 20 °C until use. Lysates were separated by electrophoresis on 4–20% gradient SurePAGE Bis–Tris gels (GeneScript) and Tris-MOPS-SDS running buffer (Cistron Script) and then transferred to PVDF membranes using Trans-Absorb Turbo Transfer Pack (Bio-Rad, Hercules, CA). The blots were probed with anti-phosphorylated EGFR (Ty1173; R&D systems), anti-phosphorylated ERK, or anti-phosphorylated AKT (Prison cell Signaling, Danvers, MA) followed by anti-Rabbit Envision+ Organization-HRP Labeled Polymer (Agilent Dako, Santa Clara, CA) and processed with Clarity™ Western ECL substrate (Bio-Rad). Point was detected using the ChemiDoc™ MP imaging system (Bio-Rad). Following stripping, the membranes were re-probed with anti-Actin mAb (MP Biomedicals, LLC, Illkirch, France). Quantitation of bands was performed using Image-Lab software (Bio-Rad).
In all figures presenting Western blots: the images in each figure are from the aforementioned experiment and treated under the same conditions (blotting, antibody hybridization exposure fourth dimension, etc.). No contrast or other visual manipulation was preformed. Where ever images contain blots that were cropped from different areas of the membrane information technology is clearly defined past dividing lines.
A Homo EGFR Phosphorylation Antibody Assortment (Abcam, Cambridge, MA) was used to notice the relative levels of 17 different phosphorylated Human EGF Receptors in cell and tissue lysates. Cell lysates were prepared and membrane treatment and probing were preformed co-ordinate to manufacturer instructions. Indicate detection and quantitation were performed using Prototype-Lab software (Bio-Rad). Bespeak intensity was normalized according to manufacturer instructions.
Flow cytometry
HF were trypsinized and immuno-stained using Alexa-488 conjugated anti-EGFR human recombinant mAb (enquiry-course Cetuximab, R&D) for xxx min on ice. Cells were washed twice in PBS, and the allowed-stained cells were analyzed past a CytoFLEX Menstruation cytometer (Beckman Coulter, Indianapolis, USA) using the CytExpert software.
RNA extraction
Total RNA was isolated from cells using Trizol reagent (Life Technologies, Paisley, UK) and purified using MaXtract High Density (QIAGEN, Redwood Urban center, CA). RNA yield and quantity were determined using a Nanodrop spectrophotometer ND-1000 (Thermo Scientific, Wilmington, DE). The RNA quality was tested using an ND-thousand V3.vii.ane, according to the manufacturer'due south instructions, and assigned an RNA integrity number (RIN). Merely samples with an RNA integrity number (RIN) of 8 or greater were employed as was previously described51.
Mig-6 mRNA expression analysis
As was previously described52 total RNA was reverse transcribed to cDNA using oligo(dT) primers and K-MLV reverse transcriptase (Thermo Scientific). PerfeCTa SYBR Green FastMix ROX (Quanta Biosciences) was used for real-time PCR co-ordinate to the manufacturer'south protocol and all the samples were run in triplicate on CFX384 Bear upon Real-Time system c1000 thermal cycler (Bio-Rad, Hercules, CA). Cycling conditions were 95 °C for 20 due south, followed past 40 cycles of 95 °C for 1 south, and sixty °C for 20 southward, 65 °C for 5 southward. Cistron expression levels were normalized to the GAPDH gene. Primers used for qPCR analysis are: Mig-6 sense-GGAGCAGTCGCAGTGAGTTT; anti-sense-GGAAGCATGCCCAAGTGGTA; GAPDH sense-AGCCTCAAGATCATCAGCAATG; anti-sense-CACGATACCAAAGTTGTCATGGAT.
RNAseq assay
Each sample is represented past three replicates of an equal amount of total RNA from 3 samples that were pooled before library construction. RNAseq libraries were synthetic in the Technion Genome Eye using Illumina TruSeq RNA Library Preparation Kit v2 according to Illumina protocol and sequenced with the Illumina HiSeq 2500 Arrangement to an average depth of around xx 1000000 reads per sample. The bioinformatics analyses were performed in the Technion Genome Centre. Raw reads were start pre-processed using trim_galore (cutadapt version 1.10) to remove adapter and low-quality sequence. The candy fastq files were mapped to the human transcriptome and genome using TopHat (v2.1.0) using Bowtie2 [version two.two.6]. The genome version was GRCh38, with annotations from Ensembl release 95. Transcript counts were quantified with HTSeq [version 0.xi.2] and differential factor expression was analyzed using the Bioconductor packet DESeq2 [version ane.24.0]. Genes with an adjusted p-value below 0.01 were considered significantly differentially expressed. Analysis for enriched pathways, upstream regulators and networks were performed using QIAGEN's Ingenuity® Pathway Assay (IPA®, QIAGEN, http://world wide web.qiagen.com/ingenuity).
Factor set enrichment analysis (GSEA53) was performed the I-CORE Bioinformatics Unit of the Hebrew University of Jerusalem and Hadassah Medical Middle for Bioinformatics data assay using whole differential expression data (cut-off independent) to determine whether a priori-defined sets of genes show statistically pregnant, concordant differences between two biological states. The authentication cistron fix collection from the molecular signatures database (MsigDB version 7.1), was used. The GEO accession number for RNAseq dataset reported in this paper is GSE183269.
Apoptosis assay
Quantification of apoptotic cells was performed using TACS Annexin V-FITC Apoptosis Detection Kit (R&D Systems) according to manufacturer instructions.
BrdU incorporation analysis
To appraise the issue of DNA harm and HB-EGF on prison cell proliferation rate, the BrdU incorporation analysis. HF were seeded and exposed to either peroxide or bleomycin and and so HB-EGF was added and cells were labeled with 10 μM of BrdU (Sigma Aldrich; MA, Us) solution for 2 h, the labeling medium was then removed and cells were incubated for an additional 24 h. Control samples were grown without BrdU supplement. At the stop of treatment cells were detached, washed and fixed using common cold accented ethanol and stored overnight at − 20 °C. For Deoxyribonucleic acid denaturation, stock-still cells were incubated for 30 min in RT with denaturation buffer (two North HCl in 0.five% triton) that was neutralized using neutralization buffer (0.1 M NaiiB4Oseven, pH8.5) and then washed with PBS. For BrdU detection, cells were incubated with xv μg/mL anti-BrdU mAb (clone BRD.three; NeoMarkers; CA, United states) Overnight at four °C and then washed, and incubated with 5 μg/mL Alexa fluor 488-conjugated F (ab′) 2 fragment goat anti-mouse IgG (Invitrogen; CA, Us) for 30 min at 4 °C.
Statistical analysis
All data were subjected to statistical assay as described52, using the Excel software package (Microsoft) or GraphPad Prism6 (GraphPad Software Inc., La Jolla, CA, USA). A 2-tailed Pupil's t-test was used to determine the difference between the groups. Data are given as mean ± SD and are shown as fault bars for all experiments. Differences were considered pregnant at P < 0.05.
References
-
Dinkelmann, Thou. et al. Multiple functions of MRN in end-joining pathways during isotype class switching. Nat. Struct. Mol. Biol. 16, 808–813. https://doi.org/10.1038/nsmb.1639 (2009).
-
Rass, Eastward. et al. Role of Mre11 in chromosomal nonhomologous end joining in mammalian cells. Nat. Struct. Mol. Biol. 16, 819–824. https://doi.org/10.1038/nsmb.1641 (2009).
-
Taylor, Due east. M. et al. The Mre11/Rad50/Nbs1 complex functions in resection-based Deoxyribonucleic acid terminate joining in Xenopus laevis. Nucleic Acids Res. 38, 441–454. https://doi.org/ten.1093/nar/gkp905 (2010).
-
Guo, X. et al. Development of a real-time PCR method for Firmicutes and Bacteroidetes in faeces and its awarding to quantify intestinal population of obese and lean pigs. Lett. Appl. Microbiol. 47, 367–373. https://doi.org/10.1111/j.1472-765X.2008.02408.x (2008).
-
Reginato, Thou. & Cejka, P. The MRE11 complex: A versatile toolkit for the repair of broken Deoxyribonucleic acid. DNA Repair 91–92, 102869. https://doi.org/x.1016/j.dnarep.2020.102869 (2020).
-
Falck, J., Coates, J. & Jackson, Due south. P. Conserved modes of recruitment of ATM, ATR and DNA-PKcs to sites of Deoxyribonucleic acid damage. Nature 434, 605–611. https://doi.org/10.1038/nature03442 (2005).
-
Stracker, T. H. & Petrini, J. H. The MRE11 complex: Starting from the ends. Nat. Rev. Mol. Prison cell Biol. 12, ninety–103. https://doi.org/x.1038/nrm3047 (2011).
-
Williams, R. S., Williams, J. S. & Tainer, J. A. Mre11-Rad50-Nbs1 is a keystone circuitous connecting Deoxyribonucleic acid repair machinery, double-strand interruption signaling, and the chromatin template. Biochem. Cell Biol. 85, 509–520. https://doi.org/10.1139/O07-069 (2007).
-
Wyman, C. & Kanaar, R. Dna double-strand break repair: All's well that ends well. Annu. Rev. Genet. xl, 363–383. https://doi.org/10.1146/annurev.genet.xl.110405.090451 (2006).
-
Fernandez-Capetillo, O., Lee, A., Nussenzweig, M. & Nussenzweig, A. H2AX: The histone guardian of the genome. Dna Repair 3, 959–967. https://doi.org/ten.1016/j.dnarep.2004.03.024 (2004).
-
Meyn, R. E., Munshi, A., Haymach, J. V., Milas, L. & Ang, K. K. Receptor signaling as a regulatory mechanism of DNA repair. Radiother. Oncol. 92, 316–322. https://doi.org/x.1016/j.radonc.2009.06.031 (2009).
-
Geiger-Maor, A. et al. Macrophages regulate the systemic response to Deoxyribonucleic acid damage by a cell not-autonomous mechanism. Cancer Res. 75, 2663–2673. https://doi.org/ten.1158/0008-5472.CAN-14-3635 (2015).
-
Schlessinger, J. Receptor tyrosine kinases: Legacy of the first two decades. Cold Spring Harb. Perspect. Biol. 6, a008912. https://doi.org/10.1101/cshperspect.a008912 (2014).
-
Yarden, Y. & Pines, Chiliad. The ERBB network: At last, cancer therapy meets systems biology. Nat. Rev. Cancer 12, 553–563. https://doi.org/10.1038/nrc3309 (2012).
-
Sabbah, D. A., Hajjo, R. & Sweidan, K. Review on epidermal growth factor receptor (EGFR) construction, signaling pathways, interactions, and recent updates of EGFR inhibitors. Curr. Elevation. Med. Chem. 20, 815–834. https://doi.org/10.2174/1568026620666200303123102 (2020).
-
Kriegs, One thousand. et al. The epidermal growth gene receptor modulates DNA double-strand interruption repair by regulating non-homologous end-joining. DNA Repair 9, 889–897 (2010).
-
Burdak-Rothkamm, S. et al. Radiosensitivity of tumor cell lines subsequently pretreatment with the EGFR tyrosine kinase inhibitor ZD1839 (Iressa). Strahlenther Onkol. 181, 197–204 (2005).
-
Mukherjee, B. et al. EGFRvIII and Deoxyribonucleic acid double-strand break repair: A molecular machinery for radioresistance in glioblastoma. Cancer Res. 69, 4252–4259 (2009).
-
Steelman, Fifty. S. et al. Therapeutic resistance in breast cancer cells can issue from deregulated EGFR signaling. Adv. Biol. Regul. 78, 100758. https://doi.org/10.1016/j.jbior.2020.100758 (2020).
-
Jost, M., Kari, C. & Rodeck, U. The EGF receptor—An essential regulator of multiple epidermal functions. Eur. J. Dermatol. 10, 505–510 (2000).
-
Chen, J., Ghorai, M. Yard., Kenney, G. & Stubbe, J. Mechanistic studies on bleomycin-mediated DNA damage: Multiple binding modes tin result in double-stranded Dna cleavage. Nucleic Acids Res. 36, 3781–3790. https://doi.org/10.1093/nar/gkn302 (2008).
-
Povirk, L. F. Dna damage and mutagenesis by radiomimetic Deoxyribonucleic acid-cleaving agents: Bleomycin, neocarzinostatin and other enediynes. Mutat. Res. 355, 71–89. https://doi.org/ten.1016/0027-5107(96)00023-1 (1996).
-
Lemmon, M. A., Schlessinger, J. & Ferguson, G. Thousand. The EGFR family unit: Not so prototypical receptor tyrosine kinases. Cold Leap Harb. Perspect. Biol. 6, a020768. https://doi.org/x.1101/cshperspect.a020768 (2014).
-
Stewart, One thousand. S. et al. The Deoxyribonucleic acid double-strand interruption repair factor hMRE11 is mutated in individuals with an clutter-telangiectasia-like disorder. Jail cell 99, 577–587. https://doi.org/10.1016/s0092-8674(00)81547-0 (1999).
-
Dupre, A. et al. A forward chemical genetic screen reveals an inhibitor of the Mre11-Rad50-Nbs1 complex. Nat. Chem. Biol. 4, 119–125. https://doi.org/10.1038/nchembio.63 (2008).
-
Shibata, A. et al. DNA double-strand suspension repair pathway choice is directed by distinct MRE11 nuclease activities. Mol. Jail cell 53, 7–18. https://doi.org/10.1016/j.molcel.2013.11.003 (2014).
-
Uziel, T. et al. Requirement of the MRN complex for ATM activation by DNA damage. EMBO J. 22, 5612–5621. https://doi.org/10.1093/emboj/cdg541 (2003).
-
Hackel, P. O., Gishizky, G. & Ullrich, A. Mig-6 is a negative regulator of the epidermal growth factor receptor signal. Biol. Chem. 382, 1649–1662. https://doi.org/x.1515/BC.2001.200 (2001).
-
Ying, H. et al. Mig-six controls EGFR trafficking and suppresses gliomagenesis. Proc. Natl. Acad. Sci. USA. 107, 6912–6917. https://doi.org/ten.1073/pnas.0914930107 (2010).
-
Li, Z. et al. Downregulation of Mig-6 in nonsmall-jail cell lung cancer is associated with EGFR signaling. Mol. Carcinog. 51, 522–534. https://doi.org/ten.1002/mc.20815 (2012).
-
Zhang, Due west. et al. The mitochondrial protein MAVS stabilizes p53 to suppress tumorigenesis. Cell Rep. thirty, 725-738.e724. https://doi.org/10.1016/j.celrep.2019.12.051 (2020).
-
Chen, J. The cell-cycle arrest and apoptotic functions of p53 in tumor initiation and progression. Common cold Bound Harb. Perspect. Med. half dozen, a026104. https://doi.org/ten.1101/cshperspect.a026104 (2016).
-
Hartwell, L. H. & Weinert, T. A. Checkpoints: Controls that ensure the social club of cell bike events. Science 246, 629–634. https://doi.org/10.1126/science.2683079 (1989).
-
Li, Y. et al. DNA impairment activates TGF-beta signaling via ATM-c-Cbl-mediated stabilization of the blazon Ii receptor TbetaRII. Jail cell Rep. 28, 735-745.e734. https://doi.org/ten.1016/j.celrep.2019.06.045 (2019).
-
Ali, R. & Wendt, Chiliad. K. The paradoxical functions of EGFR during breast cancer progression. Signal Transduct. Target Ther. 2, 1–7. https://doi.org/10.1038/sigtrans.2016.42 (2017).
-
Choi, J. et al. Epidermal growth gene induces prison cell death in the absence of overexpressed epidermal growth factor receptor and ErbB2 in various human cancer cell lines. Cancer Investig. 28, 505–514. https://doi.org/10.3109/07357900902783179 (2010).
-
Jackson, N. One thousand. & Ceresa, B. P. Protein kinase G facilitates EGFR-mediated prison cell expiry in MDA-MB-468 cells. Exp. Cell Res. 346, 224–232. https://doi.org/10.1016/j.yexcr.2016.07.001 (2016).
-
Lim, Y. J., Jeon, S. R., Koh, J. M. & Wu, H. G. Tumor growth suppression and enhanced radioresponse past an exogenous epidermal growth factor in mouse xenograft models with A431 cells. Cancer Res. Care for. 47, 921–930. https://doi.org/10.4143/crt.2014.153 (2015).
-
Wendt, M. K. et al. The antitumorigenic function of EGFR in metastatic chest cancer is regulated by expression of Mig6. Neoplasia 17, 124–133. https://doi.org/10.1016/j.neo.2014.11.009 (2015).
-
Ali, R., Dark-brown, W., Purdy, S. C., Davisson, V. J. & Wendt, G. Thousand. Biased signaling downstream of epidermal growth factor receptor regulates proliferative versus apoptotic response to ligand. Cell Death Dis. 9, 976. https://doi.org/10.1038/s41419-018-1034-vii (2018).
-
Anastasi, S., Lamberti, D., Alema, S. & Segatto, O. Regulation of the ErbB network by the MIG6 feedback loop in physiology, tumor suppression and responses to oncogene-targeted therapeutics. Semin. Prison cell Dev. Biol. 50, 115–124. https://doi.org/x.1016/j.semcdb.2015.10.001 (2016).
-
Frosi, Y. et al. A two-tiered mechanism of EGFR inhibition past RALT/MIG6 via kinase suppression and receptor degradation. J. Cell Biol. 189, 557–571. https://doi.org/10.1083/jcb.201002032 (2010).
-
Walsh, A. M. & Lazzara, M. J. Regulation of EGFR trafficking and prison cell signaling by Sprouty2 and MIG6 in lung cancer cells. J. Cell Sci. 126, 4339–4348. https://doi.org/10.1242/jcs.123208 (2013).
-
Bian, Fifty., Meng, Y., Zhang, Thou. & Li, D. MRE11-RAD50-NBS1 complex alterations and Deoxyribonucleic acid damage response: Implications for cancer treatment. Mol. Cancer 18, 169. https://doi.org/ten.1186/s12943-019-1100-5 (2019).
-
Syed, A. & Tainer, J. A. The MRE11-RAD50-NBS1 complex conducts the orchestration of damage signaling and outcomes to stress in DNA replication and repair. Annu. Rev. Biochem. 87, 263–294. https://doi.org/10.1146/annurev-biochem-062917-012415 (2018).
-
Elkon, R. et al. Dissection of a DNA-impairment-induced transcriptional network using a combination of microarrays, RNA interference and computational promoter analysis. Genome Biol. half dozen, R43. https://doi.org/10.1186/gb-2005-6-5-r43 (2005).
-
Silva, East. & Ideker, T. Transcriptional responses to DNA damage. DNA Repair 79, 40–49. https://doi.org/10.1016/j.dnarep.2019.05.002 (2019).
-
Vitelli, V. et al. Recent advancements in DNA damage-transcription crosstalk and high-resolution mapping of DNA breaks. Annu. Rev. Genomics Hum. Genet. 18, 87–113. https://doi.org/10.1146/annurev-genom-091416-035314 (2017).
-
Keating, Yard. E., Gueven, N., Watters, D., Rodemann, H. P. & Lavin, M. F. Transcriptional downregulation of ATM past EGF is defective in ataxia-telangiectasia cells expressing mutant poly peptide. Oncogene twenty, 4281–4290. https://doi.org/10.1038/sj.onc.1204527 (2001).
-
Yang, D. Q. & Kastan, M. B. Participation of ATM in insulin signalling through phosphorylation of eIF-4E-binding protein 1. Nat. Prison cell Biol. 2, 893–898. https://doi.org/x.1038/35046542 (2000).
-
Guedj, A., Geiger-Maor, A., Galun, E., Amsalem, H. & Rachmilewitz, J. Early age refuse in DNA repair capacity in the liver: In depth profile of differential gene expression. Aging 8, 3131–3146. https://doi.org/10.18632/crumbling.101120 (2016).
-
Guedj, A. et al. Gut microbiota shape "inflamm-ageing" cytokines and account for historic period-dependent refuse in Dna damage repair. Gut 69, 1064–1075. https://doi.org/10.1136/gutjnl-2019-318491 (2020).
-
Subramanian, A. et al. Cistron ready enrichment assay: A noesis-based arroyo for interpreting genome-broad expression profiles. Proc. Natl. Acad. Sci. Us 102, 15545–15550. https://doi.org/10.1073/pnas.0506580102 (2005).
Acknowledgements
Nosotros thank Dr. Chiliad. Zamir from the Department of Surgery, Hadassah Hebrew-University Medical Center, Jerusalem, Israel, for providing the primary normal human dermal fibroblasts (HF). Sequencing, quality control, and differential expression analyses were conducted by the "Technion Genome Eye". We would also like to thank Sharona Elgavish and Hadar Benyamini from the I-Core Bioinformatics Unit of the Hebrew Academy of Jerusalem and Hadassah Medical Eye for Bioinformatics data analysis. We would like to give thanks Dr. F. Rosl for critical discussions and reading of the manuscript. Special thanks to the late Dr. Ulrich Rodeck for his friendship and critical discussions. This work was supported in role by the Cooperation Programme in Cancer Research of the Deutsches Krebsforschungszentrum (DKFZ) and Israel'due south Ministry of Science, Engineering science and Infinite (MOST). Y. Five. was supported past ERC advance—GA No. 786575—RxmiRcanceR. East.G. was supported by grants from the NIH CA197081-02, the MOST, the ISF collaboration with Canada (2473/2017), the personal ISF (486/2017 and the ISF and ICORE—(ISF 41/2011), the Deutsche Forschungsgemeinschaft, Bonn, Deutschland (SFB841) and the ERC advance—GA No. 786575—RxmiRcanceR (EG). The piece of work of EG was besides supported by the Kron, Raskin and the Robert Benson foundations.
Author data
Affiliations
Contributions
Y.V. performed all the experiments under this study. R.H. performed the RNAseq experiments and assay. E.G. contributed to experimental design and data assay. J.R. designed the research, analyzed the data and wrote the manuscript.
Respective writer
Ideals declarations
Competing interests
The authors declare no competing interests.
Boosted information
Publisher'due south note
Springer Nature remains neutral with regard to jurisdictional claims in published maps and institutional affiliations.
Supplementary Information
Rights and permissions
Open Access This article is licensed under a Creative Eatables Attribution 4.0 International License, which permits use, sharing, adaptation, distribution and reproduction in any medium or format, as long as y'all give appropriate credit to the original author(due south) and the source, provide a link to the Creative Commons licence, and indicate if changes were made. The images or other third party material in this commodity are included in the commodity's Creative Commons licence, unless indicated otherwise in a credit line to the material. If material is not included in the commodity'southward Artistic Commons licence and your intended employ is not permitted by statutory regulation or exceeds the permitted use, you volition need to obtain permission straight from the copyright holder. To view a copy of this licence, visit http://creativecommons.org/licenses/by/4.0/.
Reprints and Permissions
About this commodity
Cite this article
Volman, Y., Hefetz, R., Galun, Due east. et al. Dna damage alters EGFR signaling and reprograms cellular response via Mre-xi. Sci Rep 12, 5760 (2022). https://doi.org/10.1038/s41598-022-09779-5
-
Received:
-
Accepted:
-
Published:
-
DOI : https://doi.org/x.1038/s41598-022-09779-5
Comments
By submitting a comment you agree to abide past our Terms and Community Guidelines. If y'all detect something calumniating or that does not comply with our terms or guidelines please flag it every bit inappropriate.
How Does The Cellular Response To Egf Result In Tissue Repair,
Source: https://www.nature.com/articles/s41598-022-09779-5
Posted by: kincaidnorted.blogspot.com
0 Response to "How Does The Cellular Response To Egf Result In Tissue Repair"
Post a Comment